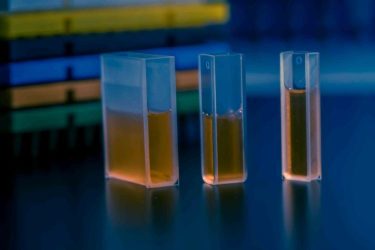
Chemometric optical microscopy techniques provide numerous opportunities for the pharmaceutic industry, both in the manufacturing stage and in R&D for drug discovery and development. One valuable application is as process analytical technology for monitoring and control of the pharmaceutical production process. Due to their ability to rapidly map the spatial distribution of active pharmaceutical ingredients (APIs) and excipients within a tablet and to identify polymorphs, they are powerful tools for online quality control and real-time feedback during the production process. Chemometric optical microscopy can also support drug discovery, being able to image the uptake, retention and metabolism of drugs inside cells in an in vivo setting and in a time lapse fashion. Such information could become crucial in the drug development process, enabling removal of ineffective compounds from the pipeline early in the discovery cycle, resulting in significant financial savings.
Fluorescence microscopy is the gold standard of optical microscopy across life sciences, with unrivalled speed and sensitivity. However, it is invasive, requiring the addition of exogenous fluorescent labels, which significantly alter the structure and function of the target biomolecules, especially small ones, as is the case for many pharmaceutical compounds. These limitations motivate the development of label-free optical microscopy techniques, which avoid the use of exogenous labels. An ideal label‑free optical microscope should: i) provide high chemical specificity, enabling identification of a material’s composition for each pixel of the image (chemometric imaging); ii) offer high spatial resolution, well below the typical size (5-10µm) of a cell; iii) enable high imaging speed for large area screening.
Some label-free optical microscopies, such as optical coherence tomography and digital holography, do not offer chemical contrast. Vibrational microscopies, on the other hand, can determine the characteristic molecular fingerprint of a sample and thus yield chemically specific information in a direct and non-destructive way. They can be separated into two complementary approaches, both suitable for chemometric imaging: infrared (IR) and Raman microscopy.1 IR microscopy directly measures the absorption of vibrational transitions in the mid-infrared range (700-3200 cm-1, corresponding to 3-14µm wavelength). The large absorption cross section of vibrational transitions results in intense signals, making IR microscopy a powerful and non-destructive chemical identification method. However, conventional Fourier transform (FT) IR microscopes that employ globars suffer from low brightness sources and noisy and expensive detectors. While the use of tunable quantum cascade lasers has improved signal‑to‑noise ratio, still light diffraction and the low numerical apertures of IR objectives limit the spatial resolution to ≈5µm, which is comparable to the size of a cell and prevents the visualisation of intra‑cellular features.
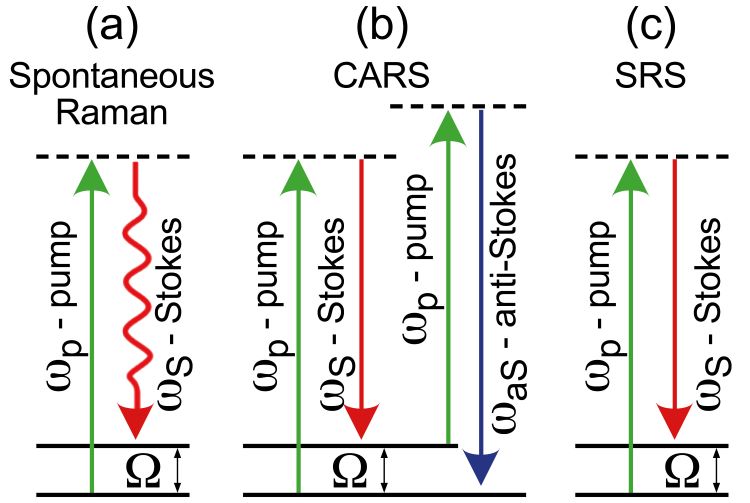
Figure 1: Scheme of the different Raman processes.
Spontaneous Raman (SR) scattering microscopy2 is a powerful and simple technique allowing both high spatial resolution (≈400nm) and excellent chemical specificity, especially when combined with chemometric analytical algorithms. In SR a monochromatic laser at frequency ωp (‘pump’) excites the sample to a virtual state, from which it relaxes to the ground state scattering photons with lower frequency ωS (‘Stokes’). The inelastic frequency shifts Ω = ωp – ωS (Figure 1a) match the frequencies of the Raman active modes, which in turn reflect the molecular structure. SR microscopy suffers from the very weak scattering cross section, due to its incoherent nature, which is 10-12 orders of magnitude lower than the cross section for fluorescence. This gives rise to low acquisition speed, with pixel dwell times of approximately one second for a Raman spectrum, resulting in measurement times of up to several hours for a high-resolution image.
Coherent Raman Scattering (CRS) microscopy3 generates the Raman signal from a coherent superposition of the vibrations in the sample, illuminated by two synchronised ultrashort laser pulses of different colour, the pump (at frequency ωp) and the Stokes (at frequency ωS). When the difference between pump and Stokes frequencies matches a vibrational frequency Ω, all the vibrational oscillators in the focal volume are resonantly excited and vibrate in phase. This vibrational coherence enhances the Raman response by many orders of magnitude with respect to the incoherent SR process, decreasing the acquisition times from seconds down to microseconds per pixel and enabling mapping of large areas with high spatial resolution. The two most widely employed CRS techniques are coherent anti-Stokes Raman scattering (CARS)4 and stimulated Raman scattering (SRS).5 In CARS (Figure 1b) the vibrational coherence is read by a further interaction with the pump beam, generating the anti‑Stokes frequency ωaS = ωp + Ω. While the CARS signal is easy to detect since its frequency differs from those of the pump and Stokes beams, it suffers from the so‑called non‑resonant background (NRB) generated by the substance under study and by the surrounding medium. The NRB does not carry any chemically specific information and distorts, and in some cases overwhelms, the resonant signal of interest. In SRS (Figure 1c) the coherent interaction with the sample induces stimulated emission from a virtual state to the investigated vibrational state, resulting in a Stokes-field amplification (stimulated Raman gain, SRG) and in a simultaneous pump-field attenuation (stimulated Raman loss, SRL). Since these signals are typically very small (10-4÷10-5 intensity variation), they are measured by high frequency modulation of the pump/Stokes beam followed by synchronous detection of the SRG/SRL with a lock-in amplifier. Despite this technical complication, SRS is the technique of choice because it is free from NRB signals and delivers a signal directly proportional to the concentration of vibrational oscillators in the focal volume.
CRS microscopies, and SRS in particular, are promising for applications to pharmaceutics, owing to their capability to obtain with high-speed chemometric maps the distribution of different components in complex heterogeneous systems. Proof‑of‑principle experiments have already been performed. Slipchenko et al. used SRS microscopy with backward (epi) detection to map with high spatial resolution the distribution of APIs and excipients in tablets of the drug amlodipine besylate, commonly employed to lower blood pressure, obtained from six different manufacturers.6 SRS microscopy imaged the uniformity of the pharmaceutical blend with high speed (104 higher than for SR microscopy) and high (sub-µm) spatial resolution. Imatinib and nilotinib are two tyrosine kinase inhibitors used for targeted cancer therapeutics. They are low molecular weight non-fluorescent molecules which are difficult to image with conventional microscopies. Fu et al. exploited the high sensitivity and speed of SRS microscopy to image the uptake and the intracellular distribution of these drugs, revealing that they accumulate outside the nuclei.7 They also performed time-lapse measurements of the drug uptake into the lysosomes, revealing the interaction of imatinib with the drug chloroquine within the cell. These results demonstrate the ability of SRS microscopy to study drug trafficking in living cells.
Until recently, SRS microscopy has been confined to specialised research laboratories due to the complexity of the required laser source. SRS in fact uses two synchronised ultrashort (few picoseconds) laser pulses at different wavelengths (the pump and the Stokes) superimposed on the sample to generate the coherent Raman signal. Current approaches use free-space solid‑state laser systems, such as an optical parametric oscillator pumped by a bulk picosecond Neodymium oscillator.8 This laser system, based on discrete, bulk optical components, has a large footprint, high cost and requires daily alignment. Nowadays this technology is being replaced by fibre lasers, which are intrinsically compact, turnkey and low cost. Suitably designed synchronised fibre lasers can generate the different colours required for SRS microscopy.9 Being fully integrated, they can be operated by non-expert users without requiring sophisticated knowledge of optics. Another limitation of SRS microscopes is that they typically work at a single frequency, requiring tuning to acquire a full vibrational spectrum. This drawback is currently overcome by broadband SRS microscopes which, employing multi‑channel lock-in amplifiers,10 acquire a full vibrational spectrum for every pixel of the image. Broadband SRS microscopes powered by fibre lasers, which are currently being developed, promise to be a game changer for high‑speed chemometric imaging. They are expected to have multiple impacts on the pharma industry, from in‑line monitoring and real-time feedback of the production process to understanding how small drug molecules interact with cells and tissues, with a great benefit for early-stage drug discovery processes.
About the authors
Giulio Cerullo, PhD
Giulio is a Full Professor with the Physics Department, Politecnico di Milano. His research activity focuses on ultrafast optics and spectroscopy and on nonlinear microscopy. He has published over 500 papers which have received over 30,000 citations (H-index 88). He is a Fellow of the Optical Society, of the European Physical Society and of the Accademia dei Lincei. He is the 2023 recipient of the EPS-QEOD Quantum Electronics Prize. He co‑founded two spinoff companies, Cambridge Raman Imaging and NIREOS.
Matteo Negro, PhD
Matteo has a PhD in Physics from Politecnico di Milano on ultrafast laser sources and spectroscopy and has worked as a Staff Researcher at the Institute of Photonics and Nanotechnologies of the Italian National Research Council (CNR-IFN). He then became Laser R&D Manager for Bios, an Italian company owned by Lumenis, a global leader in medical lasers. In 2020 he joined Cambridge Raman Imaging where he is Chief Executive and Chief Technology Officer.
References
1. Vanna R, De la Cadena A, Talone B, et al. Vibrational imaging for label-free cancer diagnosis and classification. La Rivista del Nuovo Cimento. 2021 Nov 22;45(2):107–87.
2. Krafft C, Schie IW, Meyer T, et al. Developments in spontaneous and coherent Raman scattering microscopic imaging for biomedical applications. Chemical Society Reviews. 2016;45(7):1819–49.
3. Cheng J-X, Xie XS. Vibrational Spectroscopic Imaging of Living Systems: An emerging platform for biology and medicine. Science. 2015 Nov 27;350(6264).
4. Zumbusch A, Holtom GR, Xie XS. Three-dimensional vibrational imaging by coherent Anti-Stokes Raman scattering. Physical Review Letters. 1999 May 17;82(20):4142–5.
5. Freudiger CW, Min W, Saar BG, et al. Label-free biomedical imaging with high sensitivity by stimulated Raman scattering microscopy. Science. 2008 Dec 19;322(5909):1857–61.
6. Slipchenko MN, Chen H, Ely DR, et al. Vibrational imaging of tablets by epi-detected stimulated Raman scattering microscopy. The Analyst. 2010;135(10):2613.
7. Fu D, Zhou J, Zhu WS, et al. Imaging the intracellular distribution of tyrosine kinase inhibitors in living cells with quantitative hyperspectral stimulated Raman scattering. Nature Chemistry. 2014 May 25;6(7):614–22.
8. Ganikhanov F, Carrasco S, Xie XS, et al. Broadly tunable dual-wavelength light source for coherent anti-Stokes Raman scattering microscopy. Opt. Lett. 2006;31:1292-1294.
9. Strale [Internet]. 2024 [cited 2024Feb]. Available from: https://www.cambridgeramanimaging.com/strale/
10. De La Cadena A, Vernuccio F, Ragni A, et al. Broadband stimulated Raman imaging based on multi-channel lock-in detection for spectral histopathology. APL Photonics. 2022;7(7):93946
The post Coherent Raman scattering microscopy: a powerful tool for pharmaceutics appeared first on European Pharmaceutical Review.